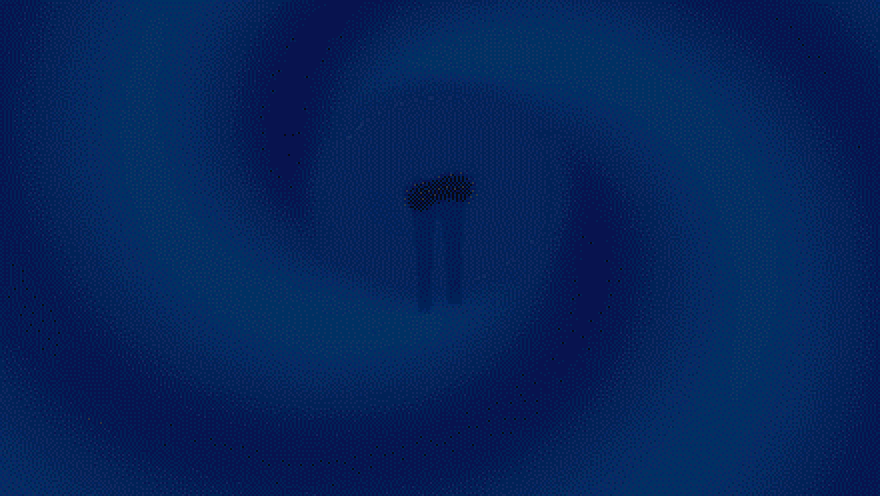
Subscribe to the Starts With a Bang newsletter
Join Dr. Ethan Siegel on a journey through the cosmos as he tackles profound questions about the universe.
Believe it or not, we are now in the year 2025, and it’s been less than ten years since the dawn of gravitational wave astronomy. Advanced LIGO, the world’s first observatory designed to detect gravitational waves, started its operations in September 2015. Within just days, it captured the first astrophysical signal from a pair of colliding black holes. As of now, we have recorded hundreds of gravitational wave events, indicative of the mergers of substantial, dense objects like black holes and neutron stars. LIGO now collaborates with additional gravitational wave observatories such as Virgo in Europe and KAGRA in Japan, enabling us to not only detect these cosmic ripples but also pinpoint their origins in the sky.
One significant event took place in 2017: the gravitational wave signal was accompanied by a gamma-ray burst detected just 1.7 seconds after the gravitational wave signal ended. This remarkable event was the result of a kilonova—showing two neutron stars colliding in a galaxy merely 140 million light-years away. Observing both electromagnetic and gravitational signals from a single astrophysical occurrence marked a turning point in multi-messenger astronomy. Then on February 6, 2025, a groundbreaking event occurred: a gravitational wave signal was linked with the detection of particles, specifically neutrinos.
This unprecedented occurrence could prove to be the most critical gravitational wave event we have ever witnessed. Here, we delve into the ongoing scientific discoveries surrounding this phenomenon.
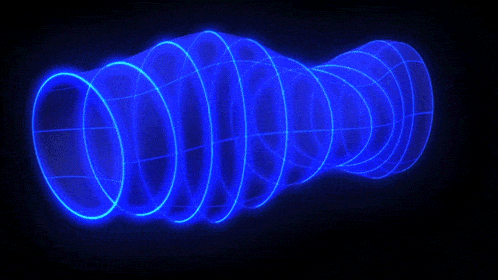
As gravitational waves traverse a region of space, they induce periodic stretching and compressing in alternate directions, leading to changes in the lengths of laser arms aligned perpendicularly. This physical phenomenon is the foundation for the successful design of gravitational wave detectors like LIGO and Virgo. It’s important to note that, unlike this simplified depiction, gravitational waves do not travel through a “tube” but rather dissipate through three-dimensional space.
The concept of multi-messenger astronomy has been established for quite some time, encompassing three distinct methods of observing the cosmos:
- The first method involves detecting light or electromagnetic signals, which is the traditional approach to astronomy using telescopes. It is noteworthy that while we primarily consider optical light visible to our eyes, most of the electromagnetic spectrum remains invisible and necessitates specialized telescopes to capture the entire range, from high-energy gamma rays to the faintest radio waves.
- The second method is particle detection, focusing on cosmic rays such as protons, nuclei, electrons, and the elusive neutrinos. Though theorized in 1930, neutrinos weren’t observed until the 1950s and have now been detected from various astrophysical sources, including the Sun and identifiable extragalactic regions like blazars.
- Finally, gravitational waves have been a consequence of Einstein’s general relativity since its inception in 1915. However, we only began to detect them directly with Advanced LIGO in 2015, inferring their existence from indirect observations prior to that.
The era of multi-messenger astronomy was not born from gravitational wave astronomy; it actually began with a supernova in a nearby galaxy, SN 1987A, in 1987.
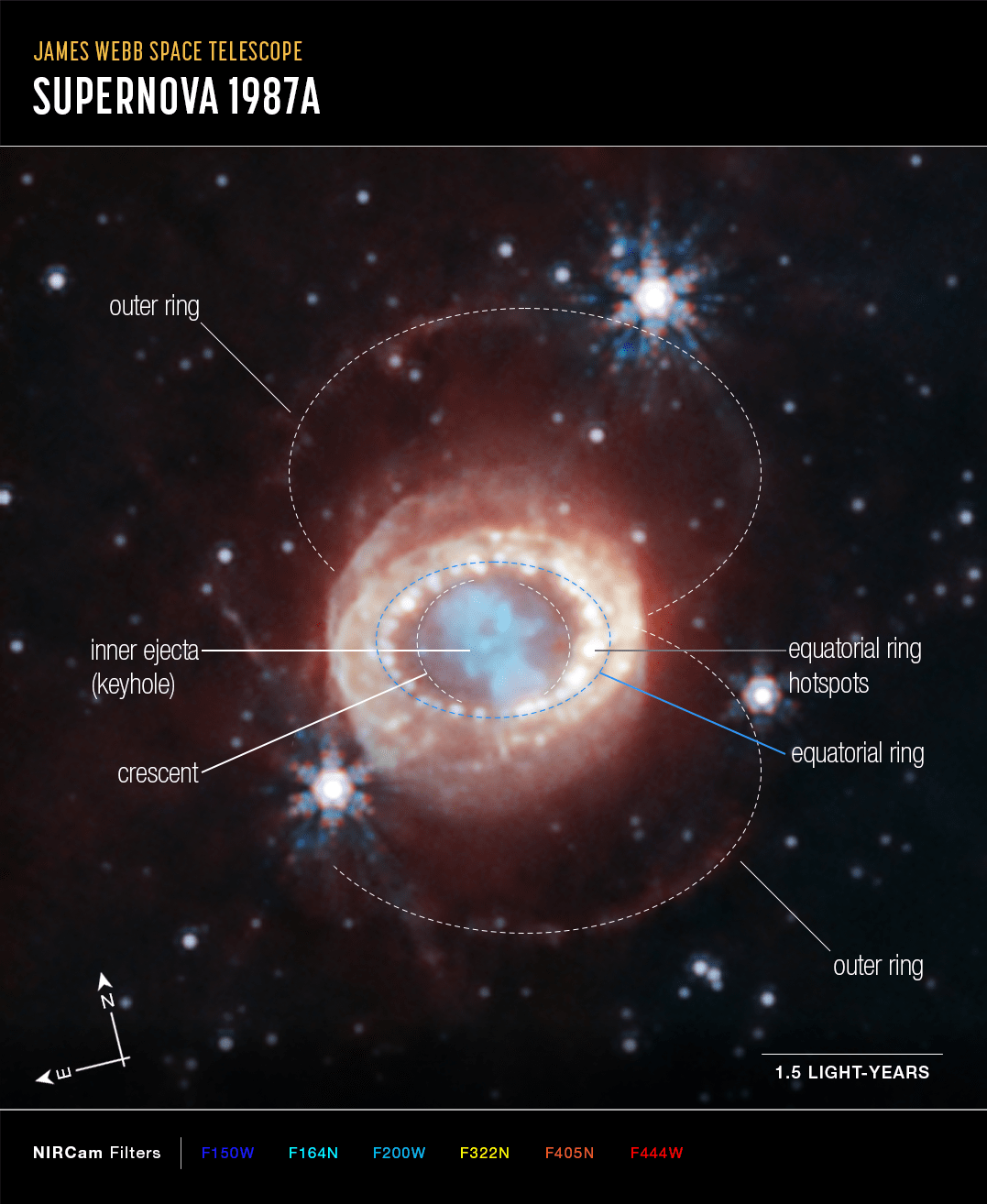
NASA’s Webb Telescope captured this enhanced image of SN 1987A. This annotated image highlights key structures— a central keyhole shape formed from ejected material from the supernova, with newly discovered faint crescents on either side and an equatorial ring consisting of material expelled long before the supernova occurred, featuring visible hotspots. Faint outer rings are visible beyond this structure.
The event known as SN 1987A was a core-collapse supernova from a galaxy nearby, approximately 165,000 light-years away, specifically the Large Magellanic Cloud. Interestingly, the first signals to arrive were not from visible light but rather a torrent of neutrinos, emitted during mere seconds in the core of the collapsing star, which were detected by existing instruments capable of measurement. This was followed by the first appearances of light several hours later, with signatures still observable today, nearly four decades later.
Since that remarkable event, no other supernova has occurred within our Local Group, limiting our ability to observe such extraordinary occurrences again. However, within this timeframe, gravitational wave detectors have been developed. Today, leading facilities like LIGO include twin interferometers in Hanford, Washington, and Livingston, Louisiana, which have made significant strides in monitoring low-mass, co-orbiting binary compact objects, allowing us to detect various mergers:
- Black hole-black hole
- Black hole-neutron star
- Neutron star-neutron star
These observations focus on pairs of objects with relatively close masses, specifically those under about 100 solar masses each.

This aerial view showcases the LIGO Livingston detector in Louisiana, viewing one of its 4 km long detector arms. Together with LIGO Hanford in eastern Washington, these facilities not only made the first detection of gravitational waves but have also contributed significantly to gravitational wave discoveries overall. Without investment in such advanced facilities, our ground-based astronomy efforts—spanning light, gravitational waves, and particle detection—would still be in their infancy.
The LIGO detectors are often regarded as the pinnacle of ground-based gravitational wave observatories and are among the most successful facilities and programs funded by the National Science Foundation. Since their inception, they have undergone multiple upgrades, employing innovative quantum technologies to enhance sensitivity. Between 2015 and 2017, the detectors recorded about ten gravitational wave events. However, since the start of their fourth (and current) data run in May 2023, which included a four-month pause from January to April 2024, they have identified a total of 193 significant detection candidates as of February 10, 2025.
Most gravitational wave events detected by LIGO (alongside Virgo and KAGRA) consist of binary black holes—remnants of large, high-mass stars that continue to orbit one another, radiating energy in the form of gravitational waves. As these orbits tighten and accelerate, they become detectable, producing signals with sufficient amplitudes and rapid frequencies. Although we can detect heavier objects from greater distances, lower-mass objects are only observable when they are relatively nearby due to mass-dependent signal amplitude.

Advanced LIGO has significantly more detection range for black hole mergers (purple) compared to neutron star mergers (yellow), illustrating the mass dependence of signal amplitude. A ~10-fold difference in range translates to nearly a ~1000-fold increase in volume. Consequently, while low-mass black holes are far more numerous, LIGO and Virgo achieve greater sensitivity for higher-mass events over more considerable distances.
On August 17, 2017, a remarkable multi-messenger event unfolded, setting the standard for future observational campaigns combining gravitational waves with other signals. Positioned just 140 million light-years away, a binary neutron star system was observed as it spiraled inward at the anticipated frequencies:
- Spiraling in at an increasingly rapid rate.
- Amtitude boosting as they neared each other.
- Finally culminating in a merger, after which the gravitational wave signal quickly dissipated.
This event alone would have been groundbreaking, representing the closest gravitational wave signal ever detected and marking the first observation of neutron stars merging through gravitational waves. However, it didn’t stand alone. Just 1.7 seconds after the gravitational wave signal ceased, NASA’s Fermi satellite detected a gamma-ray burst emanating from the same region, NGC 4993. An array of follow-up observations from various observatories on and off Earth helped astronomers piece together the event as a kilonova, a neutron star merger, a gamma-ray burst, and a site of heavy element production.

Shortly after both the gravitational wave and gamma-ray signals appeared, optical telescopes were able to focus on the galaxy housing the merger, observing the subsequent brightening and fading of the site in near real-time. The 2017 event enabled us to draw stringent limits on alternative theories of both gravity and electromagnetism, particularly given that the gamma-ray signals arrived only 1.7 seconds after the gravitational wave signal over a distance of ~130 million light-years.
From this single multi-messenger occurrence, the wealth of knowledge gained about the universe was unprecedented. Yet, despite significant advancements in our gravitational wave detection capabilities and nearing 300 confirmed gravitational wave events, very few multi-messenger gravitational wave events have been observed since.
While additional neutron star-neutron star mergers have been recorded, their distances and combined masses far exceed those seen during the 2017 event. This could mean they occurred too far away to capture their electromagnetic counterparts, or they may not have produced a kilonova since they collapsed directly into black holes instead of forming a neutron star remnant.
Neutron star-black hole mergers have been identified as well, but again, none have produced accompanying electromagnetic or particle signals. It’s possible they too went directly to black hole formation without generating detectable signals that could be observed across light or particle-detection systems.

Every neutron star merger creates a gravitational wave signal. However, whether these mergers produce an electromagnetic signal depends on multiple factors, particularly mass. When an electromagnetic signal is produced, it does so slightly after the gravitational waves.
Nonetheless, the ultimate aim of multi-messenger astronomy lies in achieving a “trifecta” event. Ideally, with sufficiently advanced observatories and a fortunate occurrence (i.e., a relevant cosmic event occurring nearby), we could detect an occurrence that yields:
- Gravitational waves
- Particles (including neutrinos)
- Electromagnetic signals (light of any wavelength)
Various astrophysical sources could fulfill this scenario, with promising candidates including:
- A neutron star-neutron star merger producing a kilonova, indicating a combined mass below approximately three solar masses
- A core-collapse supernova occurring in an asymmetric system, such as a massive star with a collapsing core orbiting closely with a stellar remnant
- A neutron star spiraling closely to a low-mass black hole, leading to tidal disruption and initiating fusion reactions (and producing detectable particles) before succumbing to the black hole’s event horizon
To realize this trifecta, we require a combination of gravitational wave detectors, a suite of telescopes covering a broad range of wavelengths, and adequately sensitive particle detectors. Neutrinos, produced abundantly during kilonovae, supernovae, and tidal disruptions, not only travel at speeds approximating that of light, but they are also exceptionally resistant to interactions with surrounding matter.
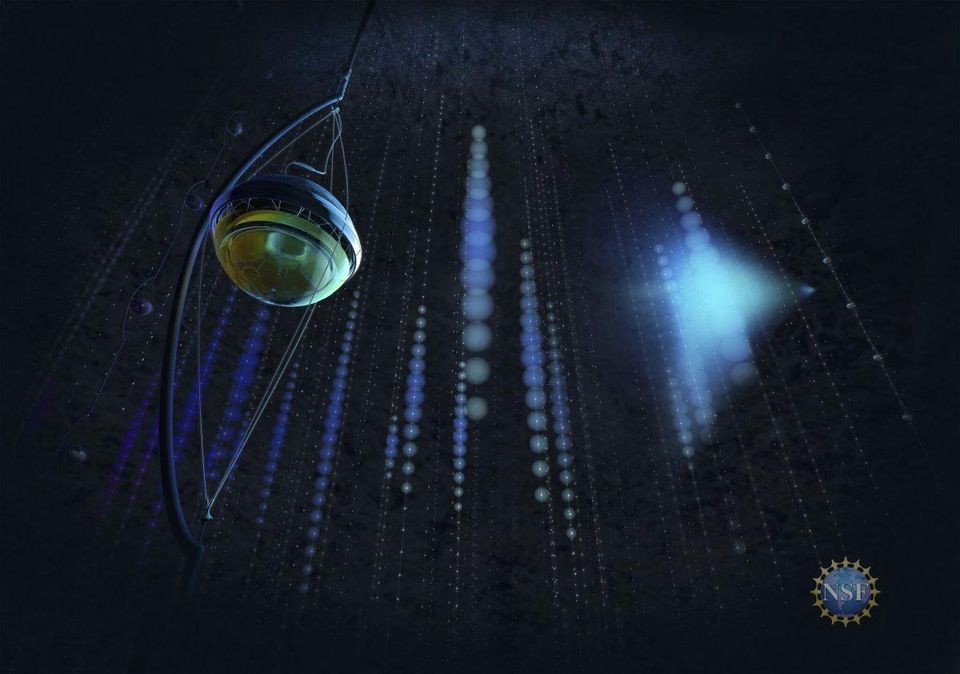
When a neutrino interacts in the clear Antarctic ice, it triggers secondary particles that create a trail of blue light as they traverse through the IceCube detector. IceCube consists of 86 strings embedded within the ice, capable of detecting the Cherenkov photons resulting from characteristic neutrino interactions. If a supernova were to occur within the Milky Way, IceCube would capture millions of neutrinos.
This is where the IceCube neutrino observatory—one of the National Science Foundation’s profound accomplishments—comes into the equation. If operational during 1987, IceCube would have detected not just a handful of neutrinos from SN 1987A but potentially millions. IceCube has also identified the most distant extragalactic neutrino signal to date, originating from a distance of 47 million light-years. In the event of a sufficiently close supernova, kilonova, or tidal disruption, IceCube could also capture neutrino signals from those occurrences.
By leveraging LIGO, IceCube, and a variety of ground-based and space-based telescopes that are sensitive to electromagnetic signals, our path to detecting the first “trifecta” multi-messenger signal hinges on the fortunate coincidence of an event happening while these facilities are functioning simultaneously.
This brings us to the present moment, specifically February 6, 2025. On this date, a significant gravitational wave event was registered: likely involving a neutron star and indicating either a neutron star-neutron star or neutron star-black hole merger, with a combined mass at the relatively low end—no more than five solar masses, and possibly as low as three solar masses.
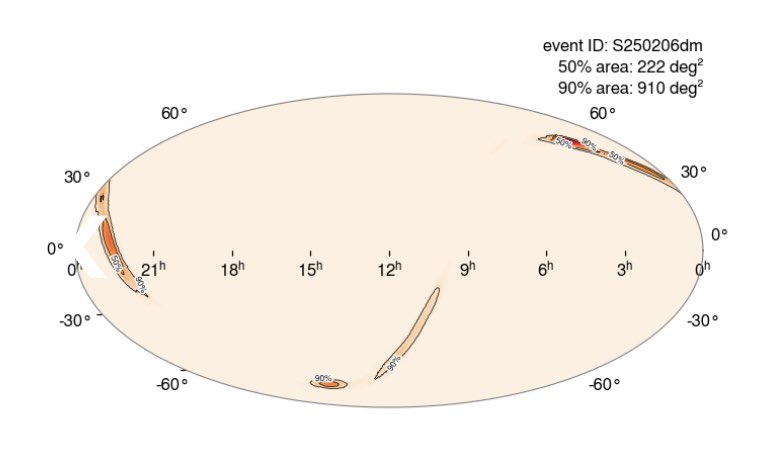
The public database of LIGO shows the sky localization parameters for gravitational wave event S250206dm, which could mark our initial multi-messenger event to feature gravitational waves alongside neutrinos and potentially an electromagnetic counterpart.
Shortly thereafter, within an hour of the automatic report on this event, the IceCube Collaboration issued its own alert, looking for possible neutrino signals occurring within 1000 seconds (approximately 17 minutes) of the gravitational wave event, consistent with the gravitational wave observations’ potential source localization. A correlation in both space and time would be a promising lead, especially since the gravitational wave event is estimated to have originated at around ~1.1 ± 0.3 billion light-years away.
Incredibly, two candidates emerged, one of which aligns exceptionally well, manifesting less than five minutes after the conclusion of the gravitational waves.
- Is it a kilonova?
- Could it be a disrupted neutron star?
- Or is it something entirely different, like a post-merger neutron star that suddenly experienced a quake or collapsed?
If confirmed, this would represent the inaugural multi-messenger event to involve both gravitational waves and particles. The central query remains: is there an associated afterglow or any form of electromagnetic signal detectable?
While detailed follow-up reports are sparse, there are whispers of something extraordinary happening: the Canadian Hydrogen Intensity Mapping Experiment (CHIME) radio telescope has detected a fast radio burst event, coinciding in time (though not necessarily in location) with the gravitational wave observation from February 6, 2025.
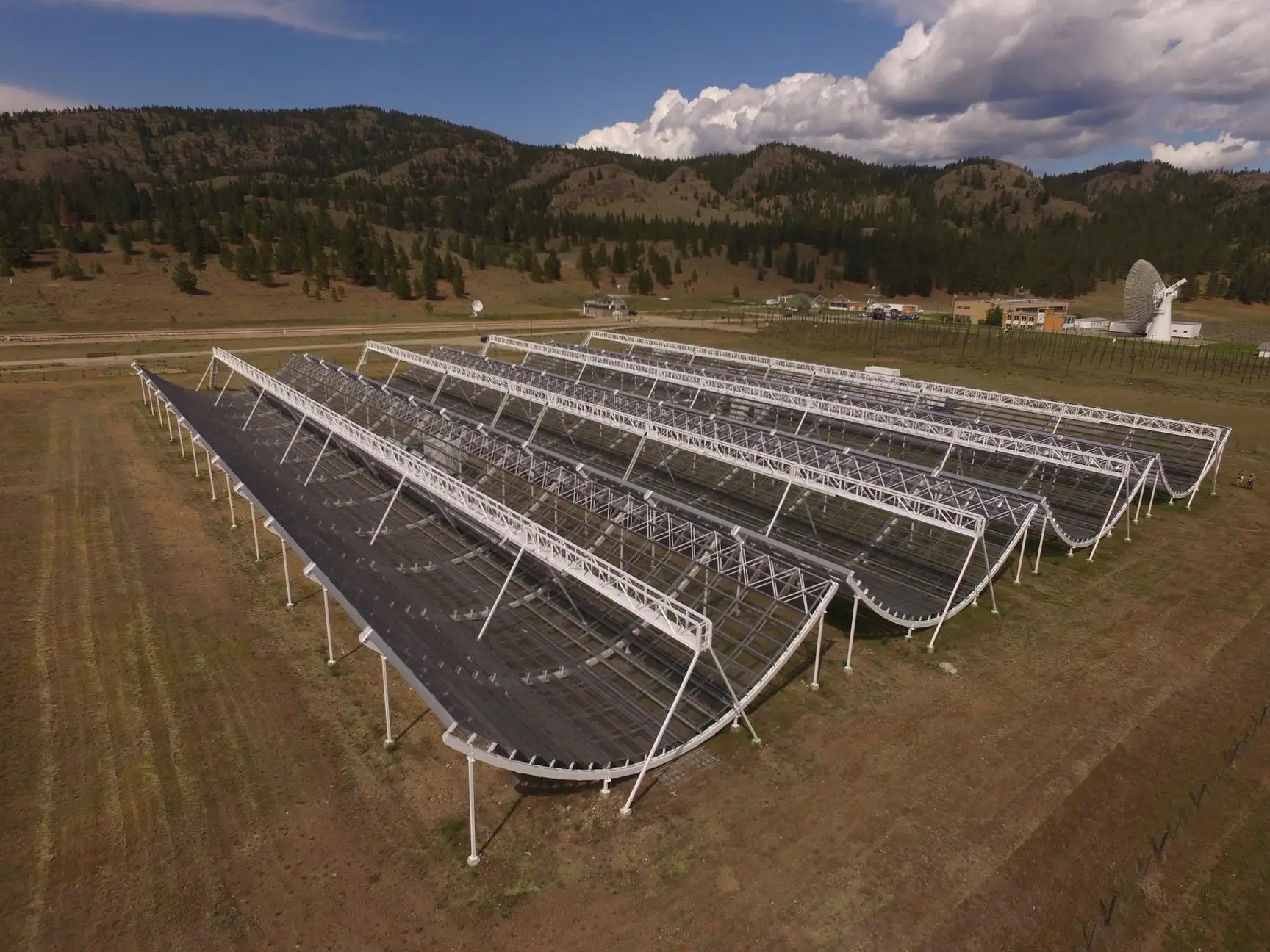
The Canadian Hydrogen Intensity Mapping Experiment, known as CHIME, recently reported a fast radio burst detected coincidentally in timing (but possibly not spatially) with the gravitational wave event and potentially the IceCube neutrino signature occurring on February 6, 2025.
If there is indeed an afterglow, or detectable remnants at any wavelength of light associated with the gravitational wave and neutrino signals from the event designated S250206dm, this would represent an unprecedented milestone in gravitational wave detection: our first trifecta event in the realm of multi-messenger astronomy. It is important to acknowledge that scientists are diligently taking their time to ensure accuracy and gather all relevant data. The complete analysis will reveal the true implications of this cosmic event for our understanding of the universe.
Furthermore, it’s crucial to note that while American scientists are calling for advanced next-generation facilities to maintain a leading position in global scientific research:
- New telescopes aimed at ushering in the era of 30-meter class ground-based observatories
- A new array of radio telescopes for improved detection of transient radio sources and monitoring pulsars for gravitational wave research
- Upgrades for the IceCube facility
- A next-generation ground-based gravitational wave detector, commonly referred to as LIGO II
There are also concerns regarding funding cuts to the National Science Foundation, which could devastate not only these scientific efforts but also put at risk the very facilities making the discovery of these monumental cosmic events possible.
We may be on the verge of witnessing what could become the most significant gravitational wave event in history. In the coming months, as data analysis continues, scientists dedicated to detecting gravitational waves, particles, and electromagnetic signals from this event will piece together its cosmic significance, providing insights into our place in the universe.
Subscribe to the Starts With a Bang newsletter
Join Dr. Ethan Siegel on a journey through the cosmos as he tackles profound questions about the universe.
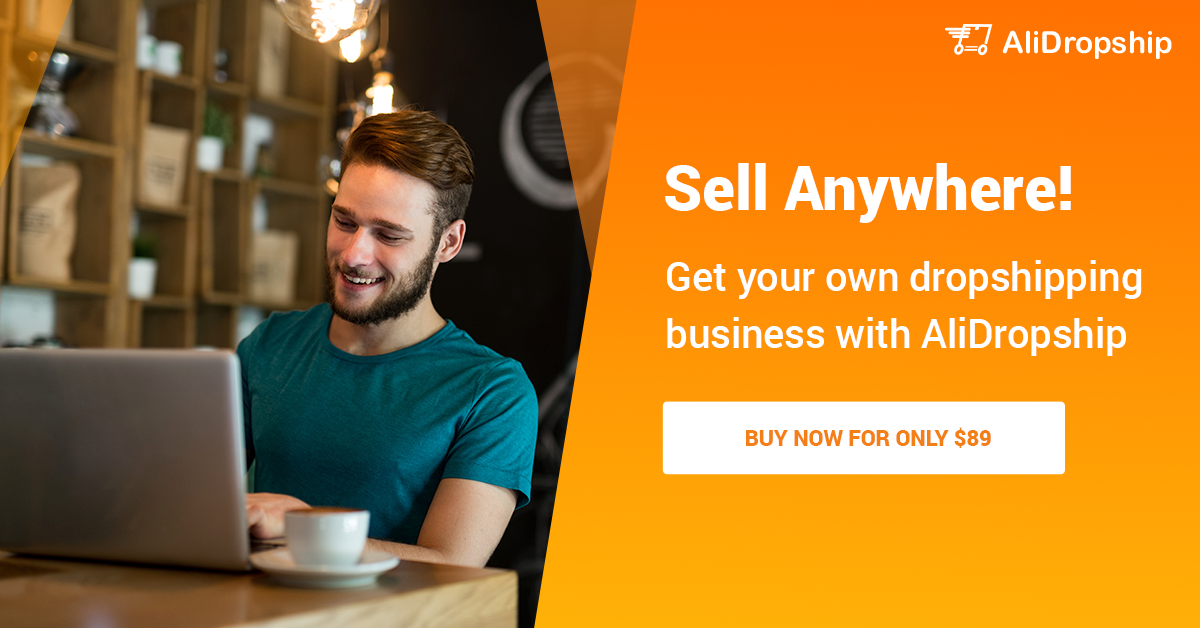