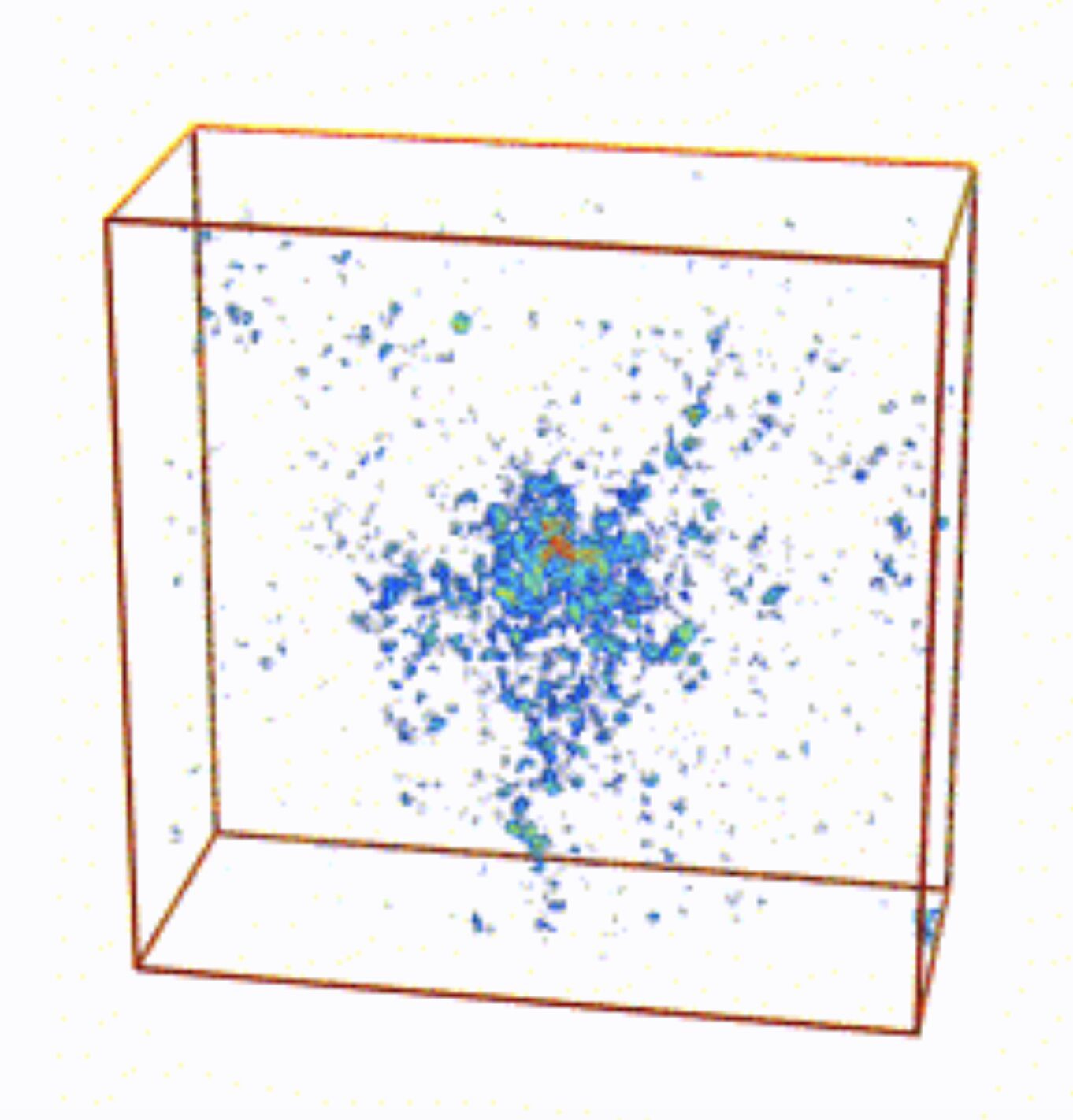

The Anderson transition refers to a phase shift occurring in disordered systems, moving from a diffusive state—where waves or particles spread out—to a localized state, where they are confined to specific regions. Initially examined by physicist Philip W. Anderson in relation to electron behavior in disordered solids, this phenomenon has also been shown to apply to the transmission of light and various other wave forms.
Recently, a team of researchers from Missouri University of Science & Technology, Yale University, and Grenoble Alpes University in France embarked on an investigation into the Anderson transition for light (i.e., electromagnetic waves) within three-dimensional disordered systems.
Their research, which has been published in the journal Physical Review Letters, details simulations of light wave transport through an arrangement of perfect-electric-conducting (PEC) spheres—materials that reflect electromagnetic waves.
“This study builds on our previous work published in Nature Physics, where we demonstrated the Anderson localization (AL) of light in 3D random media,” explained Alexey Yamilov, the primary author, to Phys.org. “However, that earlier research did not explore how the transition from diffusion to localization unfolds.”
Previous investigations into the Anderson transition have included numerical models for electron behavior in disordered materials, mechanical vibrations, and experiments with ultrasound and matter waves in cold-atom systems. These studies highlighted the universality of the transition by confirming that certain properties behave similarly across different types of physical systems.
Yamilov and his colleagues, Hui Cao and Sergey Skipetrov, aimed to illustrate that the Anderson transition for light parallels those observed in other wave types. Their first goal was to ascertain whether the observed crossover from diffusion to localization in their examined medium constituted a true Anderson transition.
They also sought to identify a critical frequency that distinguishes the two regimes (diffusion and localization), known as a sharp mobility edge.
Ultimately, they aimed to investigate how the Anderson transition demonstrates universal scaling behavior predicted by localization theory, thereby validating its universality.
“After nearly four decades of searches, our Nature Physics publication brought these inquiries to the forefront,” stated Skipetrov.
Exploring the Anderson transition poses the same fundamental challenges as studying any phase transition: it requires an infinite system, which is rarely the case in practical experiments or numerical simulations. In finite systems, what would be a sharp transition appears as a gradual crossover.
To tackle the difficulties encountered in previous phase transition studies, the researchers applied a finite-size scaling methodology. This technique allowed them to investigate the transition between diffusion and localization as the size of a 3D system increased.
“Standard statistical physics methods for analyzing phase transitions can extract transition parameters from the size-dependence observed in simulations,” stated Yamilov.
“While powerful, this method requires the ability to simulate light scattering in reasonably large (though not infinite) samples—something made possible through the innovative software Tidy3D, developed by FlexCompute, Inc.”

Leveraging Tidy3D, a comprehensive software platform for simulating electromagnetic wave behavior, the team investigated how light transmission varies with the size of a 3D metallic system at various frequencies near the Anderson transition point.
During their simulations, the researchers transmitted light pulses through metallic structures and measured the extent to which light is transmitted. This allowed them to pinpoint the frequency at which the diffusion-to-localization transition occurs, known as the critical point.
“At this significant juncture, transmission curves for various system sizes all intersect,” Yamilov described.
“The crucial factor was initiating with a system that exhibited Anderson localization of light, as reported previously. Using advanced computational techniques, we simulated large enough systems to definitively affirm that this represents a genuine phase transition, akin to that observed with electrons in disordered metals.”
The simulations conducted by Yamilov and his collaborators revealed that the transition for light within their 3D disordered systems conforms to the same universality class as other Anderson transitions. This suggests that, despite being more complex than electronic waves, electromagnetic waves share fundamental behaviors when experiencing localization.
“Until recently, creating 3D systems sufficiently large enough to observe the Anderson localization of light was unfeasible due to computational limits,” Yamilov remarked.
“Recent improvements in Finite Difference Time Domain (FDTD) algorithms from FlexCompute Inc have now made such simulations possible. This work is now accessible to anyone! In fact, our code is freely available, with FlexCompute Inc offering access for anyone interested in reviewing our results.”
Symmetry plays a crucial role in physics, with numerous previous studies demonstrating that specific properties of physical systems directly arise from their symmetries, such as time-reversal or spin-rotation symmetries, enabling straightforward predictions. The insights gained by Yamilov and his collaborators further exemplify the significance of these symmetries.
“The optical system under consideration possesses time-reversal symmetry, and only the translational symmetry is broken due to disorder,” Skipetrov clarified. “This situates the Anderson transition within the orthogonal universality class, analogous to electrons in disordered metals and vibrations in solids.”
“Our quantitative analysis of scaling behavior near the critical point yielded the first estimate of the critical exponent at approximately 1.5. This value suggests that the transition belongs to the orthogonal universality category, unveiling a profound connection between light localization and other wave phenomena, such as those involving electrons or sound, in disordered systems.”
This recent research opens exciting avenues for future exploration focusing on the manipulation of light in 3D environments. The capacity to confine light in random structures, as demonstrated in these simulations, may lead to innovative technologies, including new optical devices, sensors, and lasers based on nanoporous metals.”
“The immediate priority is to experimentally verify Anderson localization of light in 3D metallic structures, although light absorption in metals presents a challenge,” Cao noted.
“Shifting to near-infrared and microwave frequencies could mitigate this absorption issue. We are also exploring extending our numerical analysis to systems with controlled absorption to assess its impact on the transition, serving as a bridge between our idealized simulations and real materials, with the potential to guide experimental designs.”
In future investigations, the research team intends to delve deeper into the Anderson localization of light in three-dimensional metallic structures and examine practical applications of this transition, particularly how localization in nanoporous metals can enhance light-matter interactions, potentially advancing photocatalysts and sensing technologies.
For more information:
Alexey Yamilov et al, Anderson Transition for Light in a Three-Dimensional Random Medium, Physical Review Letters (2025). DOI: 10.1103/PhysRevLett.134.046302. On arXiv: DOI: 10.48550/arxiv.2408.04853
© 2025 Science X Network
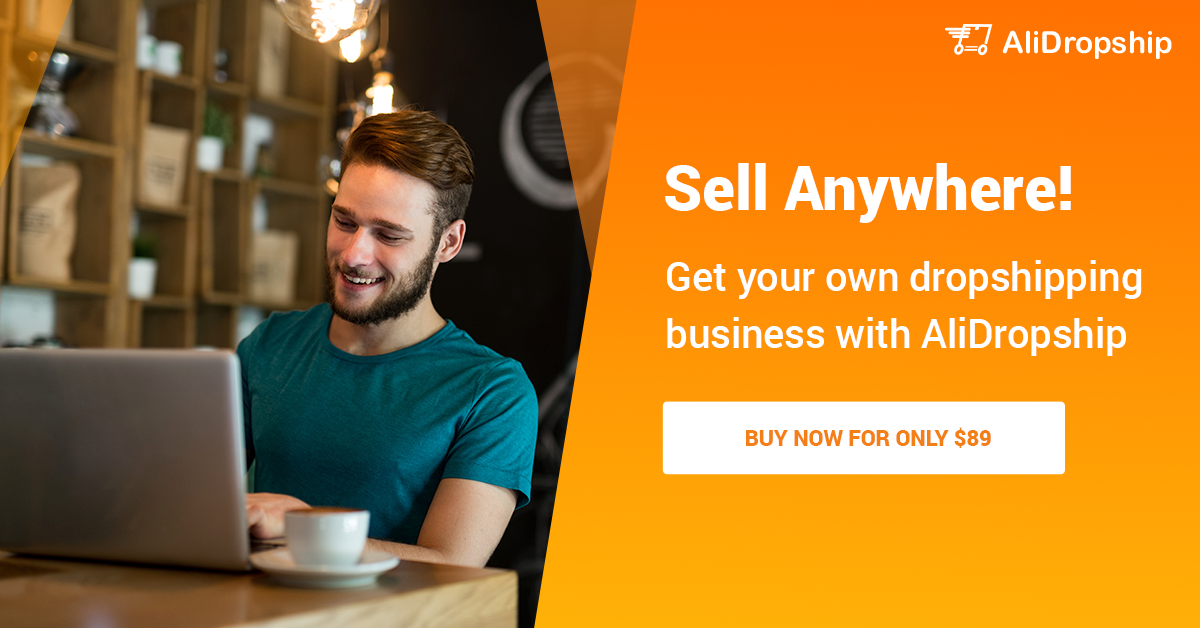